VV extracorporeal life support for the Third Millennium: will we need anticoagulation?
Introduction
The circulation of blood outside of the body was first entertained as far back as 1693 when Jean Baptiste Denis performed experiments cross-transfusing the blood of a human with “the gentle humours of a lamb” in an attempt to cure an illness in a nobleman. By 1953, John Gibbon and his wife Mary through their development of the first cardiopulmonary bypass (CPB) machine the circulation and oxygenation of blood outside the body was realized when a young lady underwent the repair of an atrial septal defect (ASD) (1). The inadvertent discovery of heparin in 1916 by Jay McLean (2) along with the characterization of coagulation and the cascade [defined by mid 1960s by the International Committee on Thrombosis and Haemostasis (ICHT) (3)] led to the ability to anticoagulate during this surgery and became the starting point to where we have evolved today in the management of patients on extracorporeal life support (ECLS).
What remains the challenge in present day is the blood biomaterial interaction and this is a barrier, which requires the use of systemic anticoagulation with or without circuit surface modifications to prevent thrombus formation within the circuitry. Unfractionated heparin (UNFH) remains the gold standard for ECLS although newer agents such as direct thrombin inhibitors (DTI) are now being considered by some as a first line option (4) and definitely in certain circumstances when UNFH cannot be used for ECLS. Technology and surface modifications continue to evolve to miniaturize ECLS devices making them more portable and biocompatible. There is no doubt in the third millennium that VV ECLS will no longer require anticoagulation and can therefore be used to support all patients who require it regardless of their acuity and risk for hemorrhage.
Present antithrombogenic measures used during ECLS
The use of ECLS is not without its challenges and complications, of which the most common are those related to hemostasis and thrombosis. The scope of this paper will focus primarily upon a brief review of present day thromboprophylaxis with a more detailed overview of where the technology should evolve to obviate the need for systemic anticoagulation during ECLS.
There is need to constantly balance the risks of bleeding against the risks of thrombosis both in the circuit and the patient; the patient’s anticoagulation must be customized for their specific pathophysiology (5). Immediately upon exposure of the patient’s blood to artificial non endothelial surfaces an inflammatory response is initiated which involves a multitude of cellular and humoral protein-driven cascades (5,6). Protein adsorption drives activation of the coagulation cascade, platelet adherence and thrombus formation. Moreover, this exposure drives stimulation of the various arms of the inflammatory apparatus including complement activation, cytokine release and production, and the recruitment of a variety of leukocytes. The activities of these, with multiple feedback loops, create a hypercoagulable state and drive the need to use systemic anticoagulation.
Systemic anticoagulation in current use
Historically the anticoagulant of choice has been UNFH. Discovered in 1916 and in clinical use since 1937, UNFH is by far the most widely used agent for systemic anticoagulation (7). In addition there is a vast and ever expanding amount of literature describing its use, its monitoring, and its effects during ECLS. For the scope and purpose of this review the details of UNFH action and monitoring will not be covered with any detail but suffice it to say that its effect is indirect, it is reliant upon the adequate presence of antithrombin, and only about 1/3 of the dose given can actually bind to antithrombin to increase antithrombin activity. The main advantages of UNFH are related to its short half-life and potential reversibility by protamine sulfate. However, it is not without complications and disadvantages ranging from unpredictable dose-response relationships, difficulty in reliable monitoring of therapeutic targets due to influence of pathophysiology, resistance to therapy (at times due to relative or absolute antithrombin deficiency) to heparin-induced thrombocytopenia (HIT) (8). Even though true HIT is probably a relatively rare event, it carries the potential for catastrophic complications.
In recent years there has been a growing volume of literature describing the use of DTIs in the context of ECLS. Recently, several excellent reviews detailing the cumulative experience in their use in this context with specific recommendation have been published (5,9,10). In general, DTI are not dependent on antithrombin for their effect but work by direct inhibition of both circulating and clot-bound thrombin—exposing the two major advantages over heparin. Moreover, their selective binding to thrombin makes their pharmacodynamics more predictable. On the downside they do not have a specific reversal agent, even though at least for bivalirudin the use of activated factor VII has been proposed as a possibility (11) and the cumulative experience with their use in this context is still sparse. Most of the literature on DTI use in ECLS relates to two commercially available products: Bivalirudin and Argatroban. Of note, in a recent survey of ECLS centers, while most responders did not use any anticoagulation other than UNFH in the months prior to answering the survey, over 50% of the responders answered that they do or can use DTI if indicated. Classic indications often reported included HIT, heparin resistance not responsive to antithrombin or FFP administration, and development of thrombosis while on heparin therapy.
Bivalirudin is a 20–amino acid synthetic polypeptide analog of hirudin, which binds to the active site of thrombin. It has a half-life of approximately 25 minutes and undergoes mostly proteolytic degradation, leaving metabolism almost independent of the liver and kidney [~20% renal elimination (12,13)], even though infusion doses should be adjusted according to renal function (4). Moreover, due to this proteolytic metabolism, in patients with cardiac standstill or in areas of stasis, anticoagulation eventually resolves and can result in thrombus formation which can lead to catastrophic results and therefore such patients might benefit from another form of anticoagulation (14,15). There have been no prospective studies examining the use of bivalirudin in patients undergoing ECLS but in the past several years two retrospective case series have been published. Ranucci et al. (4) reviewed 21 patients (9 children) on ECLS after cardiac operations and reported significantly more bleeding transfusion requirement in the heparin group while another retrospective study (16) reported no significant differences between bleeding or thrombosis. Both groups concluded that DTIs are a potential alternative to heparin in patients on ECLS, with one of the groups reporting a switch from heparin to bivalirudin as the first choice for anticoagulation in patients post cardiotomy. Specifically in children a retrospective case-series reporting on 12 patients was published by Nagle et al. (17) showing that bivalirudin can be safely used in neonates and children with maintenance dose ranges reflecting considerable inter-patient variability.
Argatroban the other common DTI, is an l-arginine derivative with a half-life similar to bivalirudin at 15 minutes; it undergoes hepatic metabolism which might represent a possible obstacle as many of the patients treated with ACLS have hepatic functional impairment. Monitoring and therapeutic targets are similar to bivalirudin as published in the ELSO anticoagulation guidelines. Its use for ECLS has been published in case reports and series for adult and pediatric patients with suspected or diagnosed HIT (18-24).
Surface modifications in current use
Current surface modifications available in clinical practice for intravascular and extracorporeal application can be divided into two major groups: biomimetic (heparin) and bio passive [phosphorylcholine (PPC), albumin and poly-2-methoxyethylacrylate (PMEA)]. Current commercially available circuitry is often a combination of both biometric capability and bio passive effect (see Table 1) (25-27).

Full table
Biomimetic surfaces
The vast majority of available data on surface-modified circuits and membranes comes from the CPB literature. In this context, the advent of heparin-bound circuits (HBC) has been first described by Gott et al. in 1963 (28). HBC have been studied extensively and have been shown to improve the biocompatibility (29-33) and perhaps the clinical outcomes (29,32,34) of extracorporeal circuits. A meta-analysis by Mangoush et al. further concluded that HBC reduce the incidence of post-operative blood transfusions, resternotomy rates, duration of ventilation, and ICU and hospital length of stay (35). A later meta-analysis, with different methodology, reconfirmed only the effect on ICU length of stay and demonstrated a reduction in atrial fibrillation events (36).
In line with the accruing evidence of HBC benefits, a recent survey has found that HBC use is on the rise (37) and in fact Bembea et al. demonstrated that over 50% of 117 ELSO (Extracorporeal Life Support Organization) centers used partial or complete HBCs. Additional studies further tested the possibility of reducing the heparinization during short CPB support using HBC with conflicting results (38,39). The main concerns regarding the use of HBC are related to the lack of effect on surface interaction and resultant worsening of thrombocytopenia (6,40). The effect of HBC on the induction of HIT is more controversial (41-43), although newer coating techniques using covalent bonding claim to obviate the leaching of heparin from the surface modification (42). Despite the binding of heparin to circuitry, the use of these circuits usually still requires some form of systemic anticoagulation so the concern of heparin leaching is only relevant when DTI is used in the presence of HIT.
Biopassive surfaces
PPC coating has been shown to be thromboresistant (44-48) and might even be noninferior to HBC in pediatric patients (49). It can also be utilized in cases of HIT where exclusion of all potential sources of heparin is needed. Furthermore, two studies by Ranucci et al. have shown that the use of PPC coating can allow a safe reduction in systemic heparinization during intraoperative ECLS (50,51). However, a prospective study on the combination of PPC and heparin coating has failed to demonstrate a significant clinical effect (52).
PMEA has also been shown to be biocompatible and might reduce the need for procedural platelet infusions in comparison to HBC (53). However, Itoh et al.have found increased incidence of post-procedural leukopenia and possibly systemic inflammatory response syndrome (SIRS) in a small prospective trial comparing HBCs to PMEA (54).
It is important to note that studies on the biocompatibility of coated circuits during ECLS are scarce. The current hypothesis on ECLS thrombogenicity states that thrombosis mechanisms during ECLS differ from those during CPB in several ways (36,42): ECLS is a closed circuit, thus activation of coagulation is related only to the blood/biomaterial interactions unlike CPB where other non-material interactions occur such as blood/air interface, hemolysis, cardiotomy suction; ECLS tends to cause more bleeding than thrombosis and its thrombogenicity can sometimes be related to the underlying condition of the patient and the support of ECLS is continued for many days/weeks with long-term exposure to the systems elements. As such, extrapolation of results from the current studies on CPB to ECLS might not be accurate. Moreover, the use of coated circuits might have an additional effect on drug adsorption during ECLS (55), although studies on the subject are scant. The final issue to consider is the extent of coating needed for circuits and whether coating the oxygenator and if present, the arterial filters are sufficient (56).
Future surface modifications to obviate systemic anticoagulation
In order to succeed with no systemic anticoagulation during ECLS the surface necessary to conquer/calm the blood/biomaterial interaction must be endothelial-like. During ECLS plasma proteins readily adsorb to the artificial surface influencing the cellular elements of the blood. More specifically it is known that fibrinogen adsorbs readily to ECLS circuits, changing its conformation thus creating a strong affinity for platelet adhesion. The end result is platelet adhesion/activation ultimately initiating coagulation. Fibrinogen is not the sole protein adsorbed obviously other epitopes which activate leucocytes also adsorb and the entire adsorption process results in the body’s response to a “foreign” surface. The endothelium is the master controller of hemostatic balance within the body. It releases both pro and anticoagulants along with anti-inflammatory agents in response to feedback loops and chemotactics. It therefore makes sense to mimic surface modifications along this path. The most recent reviews of hemocompatibility strategies in the modification of artificial surfaces range from 1993 to 2011 (57-62). There is no doubt many groups continue to research in this arena and surface modifications essentially fall within three classifications of development: surface passivation, biomimetic surface functionalization, and endothelialization of the blood contacting surfaces (Figure 1). As described above biopassive surface modifications and biomimetic surface modifications are already in clinical practice. For the purposes of this section the focus will be on new biomimetic strategies for surface modification as well as the challenges of endothelialization of artificial surfaces
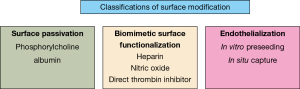
Future biomimetic surface modifications
Presently all focus on anticoagulation during ECLS is related to thrombin inhibition and manipulation of the coagulation cascade. Despite this platelet consumption continues and thrombotic events are not completely eradicated. If the focus was to be redirected to platelet inhibition however, then understanding the endothelium’s role in active platelet inhibition by the local release of nitric oxide (NO) is imperative. NO has a near complete yet fully reversible and short-lived effect on platelets. This effect is fully reversible within milliseconds once the platelet is no longer exposed to the NO and the platelet’s function is preserved in its entirety (63). Prevention of platelet activation during passage through the extracorporeal circuit is the basis upon which NO donors such as N-diazeniumdiolates have been developed. These materials are designed to be incorporated into the backbone of the polymer by either blending them into the matrix or by modifying their polymer structure to incorporate the NO within it (64-70). This prevents the leaching of the materials from the surface yet upon contact with water vapor there is local release of the NO. This local release only affects platelets directly in contact with the artificial surface while the rest remain unaffected. Thus normal hemostatic response is maintained with no systemic effects. These surface modified circuits are able to reduce platelet consumption, preserve platelet activity and reduce thrombus formation with the extracorporeal circuit in experimental models of extracorporeal circulation (71). With the ability to control NO release by chemical manipulation of the pH environment, they also have longevity despite a finite reservoir of NO within the circuit. With further study of NO releasing surfaces, it has become evident that these surfaces have an affinity for fibrinogen adsorption and thus although platelet function and number are well preserved, fibrinogen consumption occurs. Most recently Yu et al. have demonstrated the ability to incorporate argatroban within the circuit (72). In combination with NO releasing modification this surface is ideal for ECLS as it preserves normal hemostasis within the patient but provides local thromboprophylaxis within the circuit). Drawbacks are as with many new bioactive modifications, they cannot undergo regular tubing manufacturing as their bioactivity is destroyed by heat thus different methods for extrusion and sterilization must be developed.
An alternative NO biomimetic surface that does not decompose with heat exposure but also can provide a local thromboprophylactic effect is one in which catalysts are impregnated into the surface to generate NO from endogenous sources, such as S-nitrosothiols (RSNOs) through reduction/oxidation reactions causing decomposition of the RSNO and NO surface release. RSNOs circulate within the blood in picomolar to nanomolar amounts (73,74). This modification eliminates the need for the finite source of NO. By incorporation of certain metal ions such as copper directly into the polymer systems, NO-releasing decomposition of the RSNOs occurs locally and continuously to prevent platelet adhesion and activation. Unfortunately there is risk for leaching of the copper with this approach and the local release is only effective as long as the endogenous source of RSNO is replete (75). More recently the approach to this has evolved to use metal-organic frameworks (MOFs). In short these are metal ions that combine with organic ligands to form multidimensional shapes. Their physical characteristics are enticing for drug-delivery systems (76-81) and specifically copper-based MOFs have demonstrated the capability to be incorporated into the polymer material without leaching and provide excellent NO generation for local antiplatelet effect. This surface modification with MOFs is safe, extrudable, sterilizable, and active even after exposure to blood making them a very desirable option for ECLS circuitry (82).
Future endothelialized surface modifications
As discussed above the most effective surface to obviate systemic anticoagulation and retain normal hemostatic function within the patient is endothelium. Work related to endothelialization of artificial surfaces has been ongoing for decades. First attempts to preseed surfaces were carried out in the early 1980s. This was initially considered for vascular grafts and cardiac assist devices but with improving techniques can now be considered for lung assist devices and therefore perhaps prolonged ECLS as well. The complexity of endothelialization is beyond the scope of this review however touching upon the basic benefits and challenges of creating such a surface modification are important to understand. The goal of endothelializing ECLS circuitry is to recreate the endothelium. This provides a quiescent durable monolayer of functional endothelial cells to carry out all the complex hemostatic and anti-inflammatory functions of the endothelium (83). The challenge is how to achieve this coating of the circuit. Presently there are two main approaches: (I) pre-endothelialization of the circuitry in vitro (83,84) or (II) in situ capture (“fallout seeding” or “self endothelialization”) of circulating endothelial progenitor cells to surfaces modified to provide a favourable environment for cellular attachment (85-87). Although enticing there are several challenges with endothelialization of such a large surface, which include creation of a functional monolayer of quiescent endothelial cells that can hold in retention on the surface in the midst of variable often hostile flow conditions. If there is a local gap between cells then this can lead to sites for platelet adhesion/activation. But more importantly than this, it is not a surface modification that can be readily available for immediate use in any patient. It requires time to develop the endothelialization of the device. There is no doubt that as tissue engineering and surface modification techniques evolve, at some point a custom endothelialized circuit will be attainable for each patient as needed, when needed.
Conclusions
Will we need systemic anticoagulation for VV ECLS in the third millenium? As this review has shown it is extremely unlikely that systemic anticoagulation will be required. We have yet to find the perfect surface modification however several are close (Table 2). The persistence of multidisciplinary teams in the areas of chemistry, bioengineering, medicine and industry has pushed the development of biocompatible devices to the forefront addressing both the successes and failures. The goal of a biomimetic, nonthrombogenic extracorporeal surface is close to being realized. Such success will change the landscape of critical care medicine allowing for more safety not just with ECLS but also for all forms of intravascular and extracorporeal devices.
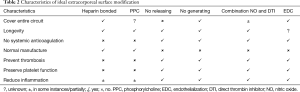
Full table
Acknowledgements
None.
Footnote
Conflicts of Interest: The authors have no conflicts of interest to declare.
References
- Fortenberry J. The history and development of extracorporeal support. In: Annich GM, Lynch WR, MacLaren G, et al., editors. ECMO: extracorporeal cardiopulmonary support in critical care. 4th edition. Ann Arbor, MI: Extracorporeal Life Support Organization, 2012:1-10.
- McLean J. The discovery of heparin. Circulation 1959;19:75-8. [Crossref] [PubMed]
- Wright IS. The nomenclature of blood clotting factors. Thrombosis et Diathesis Haemorrhagica 1962;7:381-8. [PubMed]
- Ranucci M, Ballotta A, Kandil H, et al. Bivalirudin-based versus conventional heparin anticoagulation for postcardiotomy extracorporeal membrane oxygenation. Crit Care 2011;15:R275. [Crossref] [PubMed]
- Coughlin MA, Bartlett RH. Anticoagulation for Extracorporeal Life Support: Direct Thrombin Inhibitors and Heparin. ASAIO J 2015;61:652-5. [Crossref] [PubMed]
- Reynolds MM, Annich GM. The artificial endothelium. Organogenesis 2011;7:42-9. [Crossref] [PubMed]
- Bembea MM, Annich G, Rycus P, et al. Variability in anticoagulation management of patients on extracorporeal membrane oxygenation: an international survey. Pediatr Crit Care Med 2013;14:e77-84. [Crossref] [PubMed]
- Bain J, Flannery AH, Flynn J, et al. Heparin induced thrombocytopenia with mechanical circulatory support devices: review of the literature and management considerations. J Thromb Thrombolysis 2017;44:76-87. [Crossref] [PubMed]
- Annich GM, Zaulan O, Neufeld M, et al. Thromboprophylaxis in Extracorporeal Circuits: Current Pharmacological Strategies and Future Directions. Am J Cardiovasc Drugs 2017;17:425-39. [Crossref] [PubMed]
- Ryerson LM, Lequier LL. Anticoagulation Management and Monitoring during Pediatric Extracorporeal Life Support: A Review of Current Issues. Front Pediatr 2016;4:67. [Crossref] [PubMed]
- Young G, Yonekawa KE, Nakagawa PA, et al. Recombinant activated factor VII effectively reverses the anticoagulant effects of heparin, enoxaparin, fondaparinux, argatroban, and bivalirudin ex vivo as measured using thromboelastography. Blood Coagul Fibrinolysis 2007;18:547-53. [Crossref] [PubMed]
- Hirsh J, O’Donnell M, Weitz JI. New anticoagulants. Blood 2005;105:453-63. [Crossref] [PubMed]
- Gladwell TD. Bivalirudin: a direct thrombin inhibitor. Clin Ther 2002;24:38-58. [Crossref] [PubMed]
- Ranucci M. Bivalirudin and post-cardiotomy ECMO: a word of caution. Crit Care 2012;16:427. [PubMed]
- Leissner KB, Ketchedjian A, Crowley R, et al. Deep hypothermic circulatory arrest and bivalirudin use in a patient with heparin-induced thrombocytopenia and antiphospholipid syndrome. J Card Surg 2007;22:78-82. [Crossref] [PubMed]
- Pieri M, Agracheva N, Bonaveglio E, et al. Bivalirudin versus heparin as an anticoagulant during extracorporeal membrane oxygenation: a case-control study. J Cardiothorac Vasc Anesth 2013;27:30-4. [Crossref] [PubMed]
- Nagle EL, Dager WE, Duby JJ, et al. Bivalirudin in pediatric patients maintained on extracorporeal life support. Pediatr Crit Care Med 2013;14:e182-8. [Crossref] [PubMed]
- Phillips MR, Khoury AI, Ashton RF, et al. The dosing and monitoring of argatroban for heparin-induced thrombocytopenia during extracorporeal membrane oxygenation: a word of caution. Anaesth Intensive Care 2014;42:97-8. [PubMed]
- Dolch ME, Frey L, Hatz R, et al. Extracorporeal membrane oxygenation bridging to lung transplant complicated by heparin-induced thrombocytopenia. Exp Clin Transplant 2010;8:329-32. [PubMed]
- Beiderlinden M, Treschan T, Gorlinger K, et al. Argatroban in extracorporeal membrane oxygenation. Artif Organs 2007;31:461-5. [Crossref] [PubMed]
- Scott LK, Grier LR, Conrad SA. Heparin-induced thrombocytopenia in a pediatric patient receiving extracorporeal membrane oxygenation managed with argatroban. Pediatr Crit Care Med 2006;7:473-5. [Crossref] [PubMed]
- Young G, Yonekawa KE, Nakagawa P, et al. Argatroban as an alternative to heparin in extracorporeal membrane oxygenation circuits. Perfusion 2004;19:283-8. [Crossref] [PubMed]
- Mejak B, Giacomuzzi C, Heller E, et al. Argatroban usage for anticoagulation for ECMO on a post-cardiac patient with heparin-induced thrombocytopenia. J Extra Corpor Technol 2004;36:178-81. [PubMed]
- Johnston N, Wait M, Huber L. Argatroban in adult extracorporeal membrane oxygenation. J Extra Corpor Technol 2002;34:281-4. [PubMed]
- Bauer A, Diez C, Schubel J, et al. Evaluation of hemodynamic and regional tissue perfusion effects of minimized extracorporeal circulation (MECC). J Extra Corpor Technol 2010;42:30-9. [PubMed]
- Korn RL, Fisher CA, Livingston ER, et al. The effects of Carmeda Bioactive Surface on human blood components during simulated extracorporeal circulation. J Thorac Cardiovasc Surg 1996;111:1073-84. [Crossref] [PubMed]
- Ereth MH, Nuttall GA, Clarke SH, et al. Biocompatibility of Trillium Biopassive Surface-coated oxygenator versus uncoated oxygenator during cardiopulmonary bypass. J Cardiothorac Vasc Anesth 2001;15:545-50; discussion 39-41. [Crossref] [PubMed]
- Gott VL, Whiffen JD, Dutton RC. Heparin Bonding on Colloidal Graphite Surfaces. Science 1963;142:1297-8. [Crossref] [PubMed]
- Jansen PG, te Velthuis H, Huybregts RA, et al. Reduced complement activation and improved postoperative performance after cardiopulmonary bypass with heparin-coated circuits. J Thorac Cardiovasc Surg 1995;110:829-34. [Crossref] [PubMed]
- Fosse E, Thelin S, Svennevig JL, et al. Duraflo II coating of cardiopulmonary bypass circuits reduces complement activation, but does not affect the release of granulocyte enzymes: a European multicentre study. Eur J Cardiothorac Surg 1997;11:320-7. [Crossref] [PubMed]
- Sohn N, Marcoux J, Mycyk T, et al. The impact of different biocompatible coated cardiopulmonary bypass circuits on inflammatory response and oxidative stress. Perfusion 2009;24:231-7. [Crossref] [PubMed]
- Mahmood S, Bilal H, Zaman M, et al. Is a fully heparin-bonded cardiopulmonary bypass circuit superior to a standard cardiopulmonary bypass circuit? Interact Cardiovasc Thorac Surg 2012;14:406-14. [Crossref] [PubMed]
- Gunaydin S, McCusker K, Sari T, et al. Clinical performance and biocompatibility of hyaluronan-based heparin-bonded extracorporeal circuits in different risk cohorts. Interact Cardiovasc Thorac Surg 2010;10:371-6. [Crossref] [PubMed]
- McCarthy PM, Yared JP, Foster RC, et al. A prospective randomized trial of Duraflo II heparin-coated circuits in cardiac reoperations. Ann Thorac Surg 1999;67:1268-73. [Crossref] [PubMed]
- Mangoush O, Purkayastha S, Haj-Yahia S, et al. Heparin-bonded circuits versus nonheparin-bonded circuits: an evaluation of their effect on clinical outcomes. Eur J Cardiothorac Surg 2007;31:1058-69. [Crossref] [PubMed]
- Ranucci M, Balduini A, Ditta A, et al. A systematic review of biocompatible cardiopulmonary bypass circuits and clinical outcome. Ann Thorac Surg 2009;87:1311-9. [Crossref] [PubMed]
- Sievert AN, Shackelford AG, McCall MM. Trends and emerging technologies in extracorporeal life support: results of the 2006 ECLS survey. J Extra Corpor Technol 2009;41:73-8. [PubMed]
- Gorman RC, Ziats N, Rao AK, et al. Surface-bound heparin fails to reduce thrombin formation during clinical cardiopulmonary bypass. J Thorac Cardiovasc Surg 1996;111:1-11; discussion-2.
- Ovrum E, Tangen G, Tollofsrud S, et al. Heparinized cardiopulmonary bypass circuits and low systemic anticoagulation: an analysis of nearly 6000 patients undergoing coronary artery bypass grafting. J Thorac Cardiovasc Surg 2011;141:1145-9. [Crossref] [PubMed]
- Salzman EW, Rosenberg RD, Smith MH, et al. Effect of heparin and heparin fractions on platelet aggregation. J Clin Invest 1980;65:64-73. [Crossref] [PubMed]
- Pappalardo F, Maj G, Scandroglio A, et al. Bioline heparin-coated ECMO with bivalirudin anticoagulation in a patient with acute heparin-induced thrombocytopenia: the immune reaction appeared to continue unabated. Perfusion 2009;24:135-7. [Crossref] [PubMed]
- Silvetti S, Koster A, Pappalardo F. Do we need heparin coating for extracorporeal membrane oxygenation? New concepts and controversial positions about coating surfaces of extracorporeal circuits. Artif Organs 2015;39:176-9. [Crossref] [PubMed]
- Koster A, Sanger S, Hansen R, et al. Prevalence and persistence of heparin/platelet factor 4 antibodies in patients with heparin coated and noncoated ventricular assist devices. ASAIO J 2000;46:319-22. [Crossref] [PubMed]
- Pieri M, Turla OG, Calabro MG, et al. A new phosphorylcholine-coated polymethylpentene oxygenator for extracorporeal membrane oxygenation: a preliminary experience. Perfusion 2013;28:132-7. [Crossref] [PubMed]
- Yu J, Lamba NM, Courtney JM, et al. Polymeric biomaterials: influence of phosphorylcholine polar groups on protein adsorption and complement activation. Int J Artif Organs 1994;17:499-504. [Crossref] [PubMed]
- von Segesser LK, Tonz M, Leskosek B, et al. Evaluation of phospholipidic surface coatings ex-vivo. Int J Artif Organs 1994;17:294-9. [Crossref] [PubMed]
- De Somer F, Francois K, van Oeveren W, et al. Phosphorylcholine coating of extracorporeal circuits provides natural protection against blood activation by the material surface. Eur J Cardiothorac Surg 2000;18:602-6. [Crossref] [PubMed]
- Pappalardo F, Della Valle P, Crescenzi G, et al. Phosphorylcholine coating may limit thrombin formation during high-risk cardiac surgery: a randomized controlled trial. Ann Thorac Surg 2006;81:886-91. [Crossref] [PubMed]
- Boning A, Scheewe J, Ivers T, et al. Phosphorylcholine or heparin coating for pediatric extracorporeal circulation causes similar biologic effects in neonates and infants. J Thorac Cardiovasc Surg 2004;127:1458-65. [Crossref] [PubMed]
- Ranucci M, Pazzaglia A, Isgro G, et al. Closed, phosphorylcholine-coated circuit and reduction of systemic heparinization for cardiopulmonary bypass: the intraoperative ECMO concept. Int J Artif Organs 2002;25:875-81. [Crossref] [PubMed]
- Ranucci M, Isgro G, Soro G, et al. Reduced systemic heparin dose with phosphorylcholine coated closed circuit in coronary operations. Int J Artif Organs 2004;27:311-9. [Crossref] [PubMed]
- Jacobs S, De Somer F, Vandenplas G, et al. Active or passive bio-coating: does it matters in extracorporeal circulation? Perfusion 2011;26:496-502. [Crossref] [PubMed]
- Hosoyama K, Ito K, Kawamoto S, et al. Poly-2-methoxyethylacrylate-coated cardiopulmonary bypass circuit can reduce transfusion of platelet products compared to heparin-coated circuit during aortic arch surgery. J Artif Organs 2016;19:233-40. [Crossref] [PubMed]
- Itoh H, Ichiba S, Ujike Y, et al. A prospective randomized trial comparing the clinical effectiveness and biocompatibility of heparin-coated circuits and PMEA-coated circuits in pediatric cardiopulmonary bypass. Perfusion 2016;31:247-54. [Crossref] [PubMed]
- Preston TJ, Ratliff TM, Gomez D, et al. Modified surface coatings and their effect on drug adsorption within the extracorporeal life support circuit. J Extra Corpor Technol 2010;42:199-202. [PubMed]
- Palatianos GM, Foroulis CN, Vassili MI, et al. A prospective, double-blind study on the efficacy of the bioline surface-heparinized extracorporeal perfusion circuit. Ann Thorac Surg 2003;76:129-35. [Crossref] [PubMed]
- Gorbet MB, Sefton MV. Biomaterial-associated thrombosis: roles of coagulation factors, complement, platelets and leukocytes. Biomaterials 2004;25:5681-703. [Crossref] [PubMed]
- Formigari R, Francalanci P, Gallo P, et al. Pathology of atrioventricular valve dysplasia. Cardiovasc Pathol 1993;2:137-44. [Crossref] [PubMed]
- Szott LM, Horbett TA. Protein interactions with surfaces: cellular responses, complement activation, and newer methods. Curr Opin Chem Biol 2011;15:677-82. [Crossref] [PubMed]
- Vogler EA, Siedlecki CA. Contact activation of blood-plasma coagulation. Biomaterials 2009;30:1857-69. [Crossref] [PubMed]
- Janvier G, Baquey C, Roth C, et al. Extracorporeal circulation, hemocompatibility, and biomaterials. Ann Thorac Surg 1996;62:1926-34. [Crossref] [PubMed]
- Chinn JA, Slack S. Biomaterials: Protein Surface Interactions. The Biomedical Engineering Handbook Boca Raton: CRC Press 2000;200:1597-608.
- Radomski M, Radomski A. Regulation of blood cell function by the endothelial cells. In: Vallance P, Webb D. editors. Vascular Endothelium in Human Physiology and Pathophysiology. Harwood Academic Publisher, 2000:95-106.
- Vaughn MW, Kuo L, Liao JC. Estimation of nitric oxide production and reaction rates in tissue by use of a mathematical model. Am J Physiol 1998;274:H2163-76. [PubMed]
- Miller MR, Megson IL. Recent developments in nitric oxide donor drugs. Br J Pharmacol 2007;151:305-21. [Crossref] [PubMed]
- Seabra AB, Durán N. Nitric oxide-releasing vehicles for biomedical applications. J Mater Chem 2010;20:1624-37. [Crossref]
- Al-Sa'doni HH, Ferro A. S-nitrosothiols as nitric oxide-donors: chemistry, biology and possible future therapeutic applications. Curr Med Chem 2004;11:2679-90. [Crossref] [PubMed]
- Lutzke A, Pegalajar-Jurado A, Neufeld BH, et al. Nitric oxide-releasing S-nitrosated derivatives of chitin and chitosan for biomedical applications. J Mater Chem B 2014;2:7449-58. [Crossref]
- Reynolds MM, Hrabie JA, Oh BK, et al. Nitric oxide releasing polyurethanes with covalently linked diazeniumdiolated secondary amines. Biomacromolecules 2006;7:987-94. [Crossref] [PubMed]
- Jen MC, Serrano MC, van Lith R, et al. Polymer‐Based Nitric Oxide Therapies: Recent Insights for Biomedical Applications. Adv Funct Mater 2012;22:239-60. [Crossref] [PubMed]
- Skrzypchak AM, Lafayette NG, Bartlett RH, et al. Effect of varying nitric oxide release to prevent platelet consumption and preserve platelet function in an in vivo model of extracorporeal circulation. Perfusion 2007;22:193-200. [Crossref] [PubMed]
- Yu J, Brisbois E, Handa H, et al. The immobilization of a direct thrombin inhibitor to a polyurethane as a nonthrombogenic surface coating for extracorporeal circulation. J Mater Chem B Mater Biol Med 2016;4:2264-72. [Crossref] [PubMed]
- Giustarini D, Milzani A, Colombo R, et al. Nitric oxide and S-nitrosothiols in human blood. Clin Chim Acta 2003;330:85-98. [Crossref] [PubMed]
- Stamler JS, Jaraki O, Osborne J, et al. Nitric oxide circulates in mammalian plasma primarily as an S-nitroso adduct of serum albumin. Proc Natl Acad Sci U S A 1992;89:7674-7. [Crossref] [PubMed]
- Major TC, Brant DO, Burney CP, et al. The hemocompatibility of a nitric oxide generating polymer that catalyzes S-nitrosothiol decomposition in an extracorporeal circulation model. Biomaterials 2011;32:5957-69. [Crossref] [PubMed]
- Furukawa H, Cordova KE, O'Keeffe M, et al. The chemistry and applications of metal-organic frameworks. Science 2013;341:1230444. [Crossref] [PubMed]
- Horcajada P, Gref R, Baati T, et al. Metal-organic frameworks in biomedicine. Chem Rev 2012;112:1232-68. [Crossref] [PubMed]
- Giménez-Marqués M, Hidalgo T, Serre C, et al. Nanostructured metal–organic frameworks and their bio-related applications. Coord Chem Rev 2016;307:342-60. [Crossref]
- Keskin S, Kızılel S. Biomedical applications of metal organic frameworks. Ind Eng Chem Res 2011;50:1799-812. [Crossref]
- McKinlay AC, Morris RE, Horcajada P, et al. BioMOFs: metal-organic frameworks for biological and medical applications. Angew Chem Int Ed Engl 2010;49:6260-6. [Crossref] [PubMed]
- Taylor-Pashow KM, Della Rocca J, Xie Z, et al. Postsynthetic modifications of iron-carboxylate nanoscale metal-organic frameworks for imaging and drug delivery. J Am Chem Soc 2009;131:14261-3. [Crossref] [PubMed]
- Neufeld MJ, Ware BR, Lutzke A, et al. Water-Stable Metal-Organic Framework/Polymer Composites Compatible with Human Hepatocytes. ACS Appl Mater Interfaces 2016;8:19343-52. [Crossref] [PubMed]
- Lelkes PI, Samet MM. Endothelialization of the luminal sac in artificial cardiac prostheses: a challenge for both biologists and engineers. J Biomech Eng 1991;113:132-42. [Crossref] [PubMed]
- Liu T, Liu S, Zhang K, et al. Endothelialization of implanted cardiovascular biomaterial surfaces: the development from in vitro to in vivo. J Biomed Mater Res A 2014;102:3754-72. [Crossref] [PubMed]
- Melchiorri AJ, Hibino N, Fisher JP. Strategies and techniques to enhance the in situ endothelialization of small-diameter biodegradable polymeric vascular grafts. Tissue Eng Part B Rev 2013;19:292-307. [Crossref] [PubMed]
- Avci-Adali M, Ziemer G, Wendel HP. Induction of EPC homing on biofunctionalized vascular grafts for rapid in vivo self-endothelialization--a review of current strategies. Biotechnol Adv 2010;28:119-29. [Crossref] [PubMed]
- Pang JH, Farhatnia Y, Godarzi F, et al. In situ endothelialization: Bioengineering considerations to translation. Small 2015;11:6248-64. [Crossref] [PubMed]