Multi-dimensional printing in thoracic surgery: current and future applications
Introduction
Ever since its introduction in the early 80s, the technology of three-dimensional (3D) printing, also known as additive manufacturing, has made rapid progress. Despite current uses being mainly limited to the management of complicated clinical cases, promising results and more widespread applications of the technology can be seen in various surgical specialties. Combined with advanced imaging, 3D printing has the potential to redefine personalized medicine and revolutionize the healthcare system (1). This article discuss the roles of 3D printing in thoracic surgery in simulation model and prosthesis, followed by a brief discussion on its advance application, future use and the challenges ahead.
3D printing
The introduction of 3D printing has radically changed the method of manufacturing models. Conventionally, engineers and producers prefer subtractive manufacturing, a construction method that involves various modelling techniques such as cutting and shaping to achieve a desired structure. In 3D printing, however, the object is produced layer by layer and added up. Such an additive manufacturing method is not only innovative, but also offers numerous advantages. It requires less manpower as the manufacturing process is almost automated, and it also has less demands on craftsmanship especially on manipulating complicated shaping tools. Furthermore, 3D printing can be seen as a more environmentally friendly solution to manufacturing that produces less waste, and has the ability to create objects with complex geometry.
Current medical tools and devices, as described in the literature, are printed using the fused deposition modelling (FDM) method. It works by extruding thermoplastics through hot nozzles up to 200 degree Celsius, however, the process of building a whole prosthesis can take many days. In the future, time is less likely to be a major concern when the newly introduced Continuous Liquid Interface Production (CLIP) is brought into clinical use. It works by controlling the balance between oxygen and UV light within a pool of photopolymer resin causing the resin to solidify allowing a continuous printing process. The CLIP method can markedly reduce the production process to minutes instead of hours or days (2), hence potentially allowing “real-time” on-table 3D printing for treatment of thoracic diseases for more tailored therapy (3).
Surgical planning
3D anatomical model printed based on the computed tomography (CT) images can greatly enhance preoperative planning. The ability to visualize directly from a handheld model allows surgeons to better appreciate the spatial relationships of the tumour and the bronchovascular structures.
In particular, the efficiency of this technology well demonstrated in pre-excisional localization of small nodule with diameter less than 2 cm. 3D customized navigational template has a high accuracy with a median localization time of 13 minutes (4). Besides, it has the potential to replace intraoperative CT scanning and thereby prevents the patient from excessive radiation exposure. Consequently, it can be considered as a better alternative of the frequently used CT guided hookwire localization.
Thus, 3D printing helps improve both surgical accuracy and efficiency in complex diseases. Using various colour patterns, the printed model could even indicate the effectiveness of neoadjuvant chemotherapy and fluorodeoxyglucose (FDG) activity (5). These “added dimensions” provide a more comprehensive picture, and reduces the chances of missing clinical irrelevant but abnormal lesions.
In the literature, 3D plastic lung models have been utilized in the planning of lung tumour resection (6) and subsegmentectomy (7). It is reported to preserve lung capacity to a greater extent by reducing some unnecessary removal of lung tissue and also minimizing access injury to surrounding respiratory muscles and structures. Together with the fissureless technique in thoracoscopic segmentectomy as brought by Akiba et al. (8), there is an additional benefit of preventing air leakage.
Improved surgical precision, clearly defining resection margin even prior to the surgical incision and reduced operation time, are just a few of the potential advantages of using 3D anatomical modelling. In light of these, there have been preoperative productions of personalized models of airway (9), artery (10) and vertebral body (11) for surgical planning lately, making the technology of 3D printing a valuable asset across many surgical specialties (Figure 1).
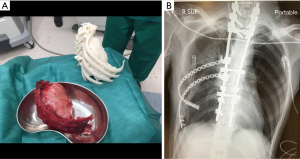
The model is also pivotal in surgical simulation. In particular, the opportunity of a hands-on practice on a realistic model before a complex procedure allows surgeons to be better prepared and identify any unforeseen challenges. Furthermore, the model could act as a platform for surgeons to practice on the controls of and manipulation of new or unfamiliar instruments prior to deploying or utilizing it on the patient (10) (Figure 2). Through practicing on the model, surgeons may have the freedom to be more inventive and develop novel surgical techniques.
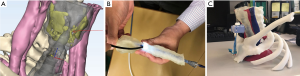
Educational purposes
Furthermore, 3D printed models would be a useful visual aid in teaching trainees, undergraduate medical students and informing the patients in complex surgical cases. Not only can surgical trainees and students appreciate distinct human anatomy, they might also gain hands-on experience on the respective procedure in simulation as discussed. In this sense, the model can also help diminish the learning curve of various operations before performing the procedure on real patients (11). Patients could also gain a better understanding of their own conditions, allowing improved decision making and communication, and ultimately better informed consent for the surgery.
Chest wall reconstruction
Various conventional chest wall reconstruction methods try to maximize the structural stability and aesthetic outcome while avoiding major acute and chronic complications such as prosthetic loosening or fracture and infection. Yet, despite recent advances in titanium prosthetic systems, they remain far from ideal (12,13). To improve prosthetic function, structural stability and aesthetics, an important factor is to create a reconstruction prosthesis that fits or even mimics the exact defect.
3D tailor made titanium ribs have quickly gained considerable popularity since its first report in 2013. The novel design even allows implants to contribute to the dynamic movement from respiratory cycles in addition to maintaining the fundamental structural integrity. Encouraging results of the 3D printed titanium ribs could be seen after its first application on a 62-year-old female patient with metastatic breast cancer invading the sternum and ribs as reported by Turna et al. in 2013 (14). The tailor-made ribs segment bears a high resemblance to the original rib curvature and could be secured to the bony remnants. Only steel wires are required to keep it in place and additional intramedullary screws added if security were a concern (15). Soft tissue reconstruction using latissimus dorsi flap and split thickness graft could be performed concomitantly depending on clinical need, for better cosmetic results. The resulting chest wall convexity and function from the printed tailor-made ribs is unlikely to be achieved by the use of mesh, or other non-customized reconstruction methods.
Recent modifications are made to maximize the functionality of the implants. Moradiellos et al. introduced a “Greek wave” folding pattern in the printed rib, adding more flexibility to the original 3D design. As a result, both the expansion and contraction phase in the respiratory cycle, can be carried out in a more physiological manner (16). A similar folding pattern can also be observed in the design employed by Aragón and Pérez Méndez (17). The “spring-like” modification on the sternocostal region as seen in both designs is also expected to reduce the risks of atelectasis due to painful breathing and eventually improving postoperative quality of life.
Nevertheless, several problem identified regarding the use of STRATOS Titanium System may draw our attention (3) in the application of 3D printed titanium ribs, particularly those with a flexible “joint” design. Metal fatigue might be one of the concerns especially when the “Greek wave” pattern is used. With the reduced density of the titanium in the sternocostal region, the trade-off of increased flexibility might be less desirable long-term outcomes. Repeated and excessive external force at that area may theoretically increase the risk of implant fracture in the long run. Furthermore, a less sturdy system may also be prone to prosthetic fracture upon direct impact, for example if the patient engages in contact sports (13).
Vertebral body reconstruction
The success and comparative advantages of 3D printing in other surgical fields have led to the concept to be revisited for spinal surgery. Significant progress has been made in the high risk procedure of vertebral reconstruction and cervical fusion (i.e., arthrodesis).
In-built pre-angulated screw holes (18-20) and porous end plates are two features shared by the 3D printed patient-specific custom vertebral body implants in the case studies. The screw holes added in the implant are made to achieve maximal stability. Xu et al., takes a step further, he added wings following the contour of the articular surface in order to create greater contact area between the implant and the vertebrae. By doing so, he is able to place additional screw on the lateral surface to further stabilize the implant and prevent dislodgement (11).
Another common characteristic seen in the customized vertebral bodies is high porosity. Porosity up to 60% enables the in-growth of bones and facilitates the process of osteointegration (20). While adding to the mechanical strength, this feature also prolongs its lifespan (21), reducing the need of revision surgery if the device is used in a young age.
Being a superior anatomical fit, 3D manufactured implants potentially saves patients from other complications if a graft is needed to be harvested from the traditional use of “off the shelf” implants or bone grafts.
Airway splint
A conservative approach with close monitoring is often adopted in mild to moderate congenital tracheomalacia. However, a consensus for management of severe tracheomalacia is lacking (22). Aortopexy is an option for experienced surgeons in selected patients, but it is ineffective among 8% of individuals, with complications encountered among 15% of patients (23). Meanwhile, the failure rate and mortality rate are reported to be higher with the use of intraluminal stents. The advancement in 3D printing may give us an alternative for handling this clinical problem.
3D airway splint is a new concept that works by suspending malfunctioning segments. While the luminal stent is placed inside the lumen; this personalized airway splint, on the contrary, is placed on the surface of the collapsed segment to achieve this effect (24). Additionally, due to its proximity with the thyroid gland and neurovascular structures, artificial patches are used to cover the scaffold, thereby acting as a cushion to prevent direct contact with delicate structures and reduce injury.
Different from the 3D prosthesis used in the chest wall or vertebral reconstruction, manufacturers of the customized tracheal splint have incorporated the use of “bioabsorbable” material polycaprolactone (PCL). The bioabsorbable material would not accumulate in the body and could be completely excreted, and this has already been tested in rat models (25). Despite the continuous degradation, the splint is designed in a fashion such that its mechanical strength would be sufficient to maintain airway patency for at least 2 years (26) before a complete resorption, which is estimated to take 3 years (27,28). The acidic environment created, in turn would promote fibrosis of surrounding tissues and also increase the strength of the malacic airway segment (29).
The effectiveness of the 3D printed tracheal splint is well demonstrated in both animal models and in real life practice. In a porcine model with surgically created life-threatening tracheobronchomalacia, placement of a 3D airway splint prolonged the survival time up to 7 days compared with the control group which succumbed in less than 1 day (26). The human case study also proves its success after implantation of an anatomically specific tracheal splint in cases of paediatric tracheobronchomalacia with no reported procedure related or short term complication (27,28).
Discussion
The current applications and the advantages that 3D printing could provide in the field of thoracic surgery are discussed, and yet there are several issues worth addressing. Foremost would be the time and cost involved, but they vary in different published case studies due to complexity, material used, and printing technology they adopt. For instance, printing a customized pulmonary artery or vertebral model may cost 700 USD (10,30), while it costs 1,500 USD (5) to create a personalized pancoast tumour model and at least 2,000 USD (30) to print the entire rib cage or thoracic spine.
Despite the high costs, there are various methods to tackle this problem. As an illustration, it is often more economical to utilize local 3D printing service than purchase a 3D printer. Depending on the model characteristics, the printing service cost may start from 100 USD per model whereas a minimum 1,500 USD is required for a decent 3D FDM printer in the market. At the higher end of 3D printer able to support multi-material printing, the cost of the machine may reach several hundred thousand USD. Other methods to save money includes using free online software such as Tinkercad or FreeCAD, attaching printed parts on a non-printed core, choosing cost-effective material such as standard resin and so forth.
Generally speaking, the most time consuming steps in processing a 3D model/implant is to design the model on the computer, which as an example, can take up to 10 hours for a pulmonary artery model (10). Moreover, the virtual models should be reviewed by a radiologist before finalizing. This collaboration would be essential given a lack of standardized guideline (30). Inaccuracies can easily occur in one of the multiple steps while processing. In light of this, a radiologist equipped with the skill of designing CAD model would be helpful in promoting 3D printing in the surgical field. This would not only shorten the processing time, but also limit the number of people handling and transferring clinical data thereby help protect patients’ privacy.
While time and cost appear to be the major obstacles in promoting the use of 3D printing in thoracic surgery, in the future, it may be compensated by the advantages addictive manufacturing offers in complex clinical scenarios. The potential reduction in operation time, fewer revision surgery and improved postoperative performance are factors that may justify the use of the expensive technology. With more documented cases and increasing experience, prospective study can be performed to validate these claimed benefits and provide an overview on long term performance.
At this juncture, there is no established regulation or protocol on manufacturing 3D implants. A regulatory framework from authorities would be necessary to limit suboptimal implants being manufactured and placed in humans. In the long term, regulations on sterilization would be useful as well. Of equal importance would be 3D printing research collaborations with other units such as tissue engineering, and regenerative medicine so as to facilitate a revolutionary advancement in the health care system (31). The following provides an update and brief discussion on two of the most exciting developments; bioprinting and 4D printing.
Beyond 3D printing: bioprinting
Bioprinting is a rapidly developing branch in regenerative medicine, aiming to reproduce cells, tissues, and ultimately, fully functional organs. A detailed review on this field is beyond the scope of this article, however, the following serves to provide a general overview and discuss its possible application.
Taking the advantages of improved precision and spatial control provided by 3D printing, great progress is made in bioprinting with a more accurate deposition of viable cells, and supporting components on a biocompatible scaffold. Nonetheless, the field is still in its infancy and many more challenges are to be met before a living tissue can be created.
Foremost, a more comprehensive knowledge and coordination between various specialities, including material science, engineering and chemistry is required. Having knowledge of the microenvironment and cell-cell interaction, for example, would determine the material chosen for a biocompatible scaffold, correlating with the cell’s functionality and viability (32). Of equal importance in facilitating bioprinting is the appropriate choice of biomaterial as BioInk, and achieving subsequent tissue vascularization for ongoing development. An ideal BioInk should be able to differentiate into a wide spectrum of cells, easily isolated and stored. Moreover, extended lifespan and adequate mechanical strength are also desirable characteristics. At present, embryonic stems cells are considered as one of the top candidates to be used as BioInk (31). After printing those functional tissues or cells, vasculature would be the key in controlling cells’ survival and maturation (33). Without continuous supply of nutrients and waste removal, tissues would undergo apoptosis. As such, further research on bioreactor supporting vasculature and angiogenesis is necessary (34).
Although an organ replica is unlikely to be achieved in short term, the technology introduced in bioprinting has a widespread application in bridging the gap between the current medical practice and the ideal personalized medicine. First and foremost, printed human tissues would facilitate pharmacological development to a great extent. Drug trials can be tested directly on human tissues instead of animal model (35), allowing more precise trials, higher safety profile, more affordability and potentially more conditions to be treated. Printed tissue samples could also provide individualized drug regimen thus speeding up the recovery. Besides, the technology, per se, is an asset in learning pathology (34). In vitro model enables direct examination of various types of conditions. For instance, a thorough understanding on the aetiology of pneumonia or pathogenesis of fibrosis is made possible after inoculation of microorganism or pollutants on human lung tissue.
Bioprinting organ for transplantation in many ways can be seen as medicine’s Holy Grail. With a more mature and well developed technology, in vivo bioprinting (31) also emerges as a promising alternative. It works by depositing cells and biological material directly on the patients. In this sense, a fibrotic diseased lung can be repaired after adding appropriate biomaterials and cells even without debridement. Multidisciplinary approaches with the input from various fields, including but not limited to engineering, medicine, biology, chemistry, would truly be necessary in getting closer to the dream of creating a 3D printed organ (35).
4D printing—the new horizon
Another fascinating area of development is the recently introduced concept of 4D printing. Incorporating the concept of “time” into conventional 3D printing, it enables the printed objects to change form and/or function when exposed to a predetermined stimulus, such as heat, pressure, or humidity (36).
Conventionally fabricated objects have a pre-designated form and modality that should remain static and stable. The new concept of 4D printing will challenge this idea, with the ability to undergo self-transformation once produced, and facilitate minimally invasive deployment thereby improving procedural cosmesis (37). Besides, 4D printing also enables a change in physical, chemical and/or molecular properties, resulting in an object with distinct flexibility, porosity, conductivity, magnetic properties and so forth. At present, shape memory polymer (38), hydrogels (39) and cross-linked liquid crystalline polymers (40) are the soft active materials (36) favoured in the 4D printing industry.
Clinically, personalized endo-luminal medical devices are the first and, at present, the only documented application of 4D printing (38). Applications include cardiovascular stent for expanding narrowed lumen (34) and airway stent composed of shape memory polymer (38). Actuation, also known as transformation, is seen when device is subjected to heat as accomplished with the use of thermal chamber, infrared diode laser, inductive and resistive heating. Apart from stents, other future devices that may benefit from such characteristics of 4D printing include paediatric rib cage implant and prosthetic cardiac valves. The advantage in the rib cage would be the added ability to accommodate physiological growth and dynamic respiratory cycle, whereas in a valve model, flow rate could be well adjusted in response to the physical demands and oxygen concentration in blood.
Another perspective of 4D printing would be the capability to undergo self-repairing. The underlying mechanism is mostly contributed by the reversibility of the cross linking at the molecular level (39). This has a vast therapeutic application in regenerative medicine. Other than reducing revision surgery in case of device migration and structural failure, outdated 4D printed devices and objects, can be decomposed into programmable particles or components for recycling. Although the idea of 4D printing is currently in the experimental phases, illustrated above are some of the potential advantages and uses. Other than clinical applications, it also provides a wide range of opportunities to be explored by various manufacturing industries.
Conclusions
3D printing is a new medical technology with potential widespread applications in thoracic surgery. Currently, the main utilizations are in printed models for surgical planning, education and implant. For the time being, the high cost and prolonged processing time may preclude mass application. Nonetheless, the development of 3D printing provides the fundamental basis for generating more exciting ideas, including bioprinting and 4D printing. With advancement in printing technology and further collaborative endeavours that can streamline and shorten the process, additive manufacturing will likely bring a paradigm shift in the planning and management of thoracic conditions in the near future.
Acknowledgements
None.
Footnote
Conflicts of Interest: The authors have no conflicts of interest to declare.
References
- Ng CS, He JX, Rocco G. Innovations and technologies in thoracic surgery. Eur J Cardiothorac Surg 2017;52:203-5. [Crossref] [PubMed]
- Tumbleston JR, Shirvanyants D, Ermoshkin N, et al. Additive Manufacturing. Continuous liquid interface production of 3D objects. Science 2015;347:1349-52. [Crossref] [PubMed]
- Ng CS. Recent and Future Developments in Chest Wall Reconstruction. Semin Thorac Cardiovasc Surg 2015;27:234-9. [Crossref] [PubMed]
- Zhang L, Li M, Li Z, et al. Three-Dimensional printing of navigational template in localization of pulmonary nodule: A pilot study. J Thorac Cardiovasc Surg 2017;154:2113-9.e7. [PubMed]
- Gillaspie EA, Matsumoto JS, Morris NE, et al. From 3-Dimensional Printing to 5-Dimensional Printing: Enhancing Thoracic Surgical Planning and Resection of Complex Tumors. Ann Thorac Surg 2016;101:1958-62. [Crossref] [PubMed]
- Kim MP, Ta AH, Ellsworth WA 4th, et al. Three dimensional model for surgical planning in resection of thoracic tumors. Int J Surg Case Rep 2015;16:127-9. [Crossref] [PubMed]
- Nakada T, Akiba T, Inagaki T, et al. Thoracoscopic anatomical subsegmentectomy of the right S2b + S3 using a 3D printing model with rapid prototyping. Interact Cardiovasc Thorac Surg 2014;19:696-8. [Crossref] [PubMed]
- Akiba T, Nakada T, Inagaki T. Simulation of the Fissureless Technique for Thoracoscopic Segmentectomy Using Rapid Prototyping. Ann Thorac Cardiovasc Surg 2015;21:84-6. [Crossref] [PubMed]
- Guibert N, Moreno B, Plat G, et al. Stenting of Complex Malignant Central-Airway Obstruction Guided by a Three-Dimensional Printed Model Of The Airways. Ann Thorac Surg 2017;103:e357-9. [Crossref] [PubMed]
- Kurenov SN, Ionita C, Sammons D, et al. Three-dimensional printing to facilitate anatomic study, device development, simulation, and planning in thoracic surgery. J Thorac Cardiovasc Surg 2015;149:973-9.e1. [Crossref] [PubMed]
- Xu W, Zhang X, Ke T, et al. 3D printing–assisted preoperative plan of pedicle screw placement for middle-upper thoracic trauma: a cohort study. BMC Musculoskelet Disord 2017;18:348. [Crossref] [PubMed]
- Ng CS, Ho AM, Lau RW, et al. Chest Wall Reconstruction with MatrixRib System-Avoiding Pitfalls. Interact Cardiovasc Thorac Surg 2014;18:402-3. [Crossref] [PubMed]
- Ng CS, Wong RH, Kwok MW, et al. Delayed Fracture of MatrixRib Pre-contoured Plate System. Interact Cardiovasc Thorac Surg 2014;19:512-4. [Crossref] [PubMed]
- Turna A, Kavakli K, Sapmaz E, et al. Reconstruction with a patient-specific titanium implant after a wide anterior chest wall resection. Interact Cardiovasc Thorac Surg 2014;18:234-6. [Crossref] [PubMed]
- Simal I, García-Casillas MA, Cerdá JA, et al. Three-Dimensional Custom-Made Titanium Ribs for Reconstruction of a Large Chest Wall Defect. European J Pediatr Surg Rep 2016;4:26-30. [Crossref] [PubMed]
- Moradiellos J, Amor S, Córdoba M, et al. Functional Chest Wall Reconstruction With a Biomechanical Three-Dimensionally Printed Implant. Ann Thorac Surg 2017;103:e389-91. [Crossref] [PubMed]
- Aragón J, Pérez Méndez I. Dynamic 3D printed titanium copy prosthesis: a novel design for large chest wall resection and reconstruction. J Thorac Dis 2016;8:E385-9. [Crossref] [PubMed]
- Choy WJ, Mobbs RJ, Wilcox B, et al. Reconstruction of Thoracic Spine Using a Personalized 3D-Printed Vertebral Body in Adolescent with T9 Primary Bone Tumor. World Neurosurg 2017;105:1032.e13-1032.e17. [Crossref] [PubMed]
- Mobbs RJ, Coughlan M, Thompson R, et al. The utility of 3D printing for surgical planning and patient-specific implant design for complex spinal pathologies: case report. J Neurosurg Spine 2017;26:513-8. [Crossref] [PubMed]
- Phan K, Sgro A, Maharaj MM, et al. Application of a 3D custom printed patient specific spinal implant for C1/2 arthrodesis. J Spine Surg 2016;2:314-8. [Crossref] [PubMed]
- Rao PJ, Pelletier MH, Walsh WR, et al. Spine Interbody Implants: Material Selection and Modification, Functionalization and Bioactivation of Surfaces to Improve Osseointegration. Orthop Surg 2014;6:81-9. [Crossref] [PubMed]
- Valerie EP, Durrant AC, Forte V, et al. A decade of using intraluminal tracheal/bronchial stents in the management of tracheomalacia and/or bronchomalacia: is it better than aortopexy? J Pediatr Surg 2005;40:904-7. [Crossref] [PubMed]
- Torre M, Carlucci M, Speggiorin S, et al. Aortopexy for the treatment of tracheomalacia in children: review of the literature. Ital J Pediatr 2012;38:62. [Crossref] [PubMed]
- An J, Chua CK. An engineering perspective on 3D printed personalized scaffolds for tracheal suspension technique. J Thorac Dis 2016;8:E1723-5. [Crossref] [PubMed]
- Sun H, Mei L, Song C, et al. The in vivo degradation, absorption and excretion of PCL-Based implant. Biomaterials 2006;27:1735-40. [Crossref] [PubMed]
- Zopf DA, Flanagan CL, Wheeler M, et al. Treatment of Severe Porcine Tracheomalacia With a 3-Dimensionally Printed, Bioresorbable, External Airway Splint. JAMA Otolaryngol Head Neck Surg 2014;140:66-71. [Crossref] [PubMed]
- Zopf DA, Hollister SJ, Nelson ME, et al. Bioresorbable Airway Splint Created with a Three-Dimensional Printer. N Engl J Med 2013;368:2043-5. [Crossref] [PubMed]
- Morrison RJ, Hollister SJ, Niedner MF, et al. Mitigation of tracheobronchomalacia with 3D-printed personalized medical devices in pediatric patients. Sci Transl Med 2015;7:285ra64. [Crossref] [PubMed]
- Huang L, Wang L, He J, et al. Tracheal suspension by using 3-dimensional printed personalized scaffold in a patient with tracheomalacia. J Thorac Dis 2016;8:3323-8. [Crossref] [PubMed]
- Giannopoulos AA, Steigner ML, George E, et al. Cardiothoracic Applications of 3-dimensional Printing. J Thorac Imaging 2016;31:253-72. [Crossref] [PubMed]
- Murphy SV, Atala A. 3D bioprinting of tissues and organs. Nat Biotechnol. 2014;32:773-85. [Crossref] [PubMed]
- Goldstein TA, Epstein CJ, Schwartz J, et al. Feasibility of Bioprinting with a Modified Desktop 3D Printer. Tissue Eng Part C Methods 2016;22:1071-6. [Crossref] [PubMed]
- Gu Q, Hao J, Lu Y, et al. Three-Dimensional bio-Printing. Sci China Life Sci 2015;58:411-9. [Crossref] [PubMed]
- Yoo SS. 3D-Printed biological organs: medical potential and patenting opportunity. Expert Opin Ther Pat 2015;25:507-11. [Crossref] [PubMed]
- Gao G, Huang Y, Schilling AF, et al. Organ Bioprinting: Are We There Yet? Adv Healthc Mater 2018.7. [PubMed]
- Campbell T, Tibbits S, Garrett B. The Next Wave: 4D Printing - Programming the Material World. Atlantic Council, Washington, Brent Scowcroft Center on International Security May 2014. Available online: http://www.atlanticcouncil.org/images/publications/The_Next_Wave_4D_Printing_Programming_the_Material_World.pdf
- Chen T, Shea K. An Autonomous Programmable Actuator and Shape Reconfigurable Structures using Bistability and Shape Memory Polymers. Cornell University Library Nov 2017. Available online: https://arxiv.org/abs/1711.00452
- Zarek M, Mansour N, Shapira S, et al. 4D Printing of Shape Memory-Based Personalized Endoluminal Medical Devices. Macromol Rapid Commun 2017.38. [PubMed]
- Wang Y, Adokoh CK, Narain R. Recent development and biomedical applications of self-healing hydrogels. Expert Opin Drug Deliv 2018;15:77-91. [Crossref] [PubMed]
- López-Valdeolivas M, Liu D, Broer DJ, et al. 4D Printed Actuators with Soft-Robotic Functions. Macromol Rapid Commun 2018.39. [PubMed]